Ready to proof -- Clare 2/7/22
KJ proofed and sent corrections on 2/7
CLJ revised on 2/7
Ready for author
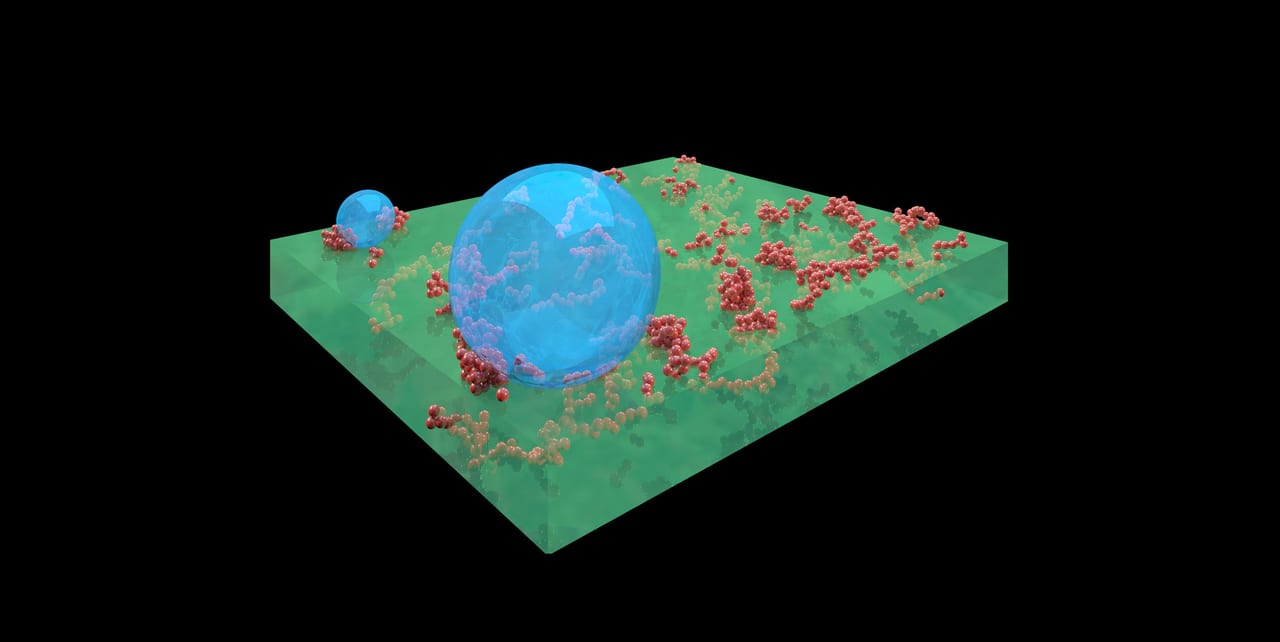
Photo courtesy of Iowa State University.
Enhancing Biobased Polymer Coatings Through Nanoparticle Assembly
By Emily Olson, Materials Science and Engineering, and Polymer and Food Protection Consortium; Rebecca Mort, Materials Science and Engineering, and Polymer and Food Protection Consortium; Yifan Li, Materials Science and Engineering; Dr. Keith Vorst, Polymer and Food Protection Consortium, and Food Science and Human Nutrition; Dr. Greg Curtzwiler, Polymer and Food Protection Consortium, and Food Science and Human Nutrition; and Dr. Shan Jiang, Materials Science and Engineering, and Polymer and Food Protection Consortium, Iowa State University, Ames, IA
The alarming volumes of plastic waste in landfills require sustainable alternatives. Biobased polymers are a promising replacement, but performance challenges limit their introduction in a competitive, cost-driven market. One approach to increase the performance and functionality of biobased polymers is through addition of nanoparticles. In this work we demonstrate that strategic assembly of nanoparticles can yield new and interesting properties to the biopolymer matrix. For example, nanoassembly can be used to design waterborne biobased polymer nanocomposites with superhydrophobicity or UV-blocking properties. Detailed characterization and computation modelling have shown that the morphology of biobased polymers plays an important role in determining the assembly structures and the ultimate performance.
Introduction
Increasing amounts of plastic waste (348 million tons in 2017)1, 2 and food waste in retail (up to 133 billion pounds and 161 billion USD),3 require green solutions immediately. Biobased polymers are attractive alternatives to petroleum polymer systems, but poor performance in terms of mechanical properties, thermal stability and water sensitivity limit their integration into petroleum-dominated industries. Nanoparticles have been widely used to enhance the performance of petroleum polymers. Interestingly, few studies address nanoparticle integration in biobased polymer systems.4, 5 Less fundamental research in biobased nanocomposites is likely a result of inherently variable sources of biobased polymers, which make the results less reproducible.6-11 Nanoparticles have been demonstrated to impart properties including antibacterial activity,12-14 corrosion resistance,15, 16 strength,17, 18 and hydrophobicity19-21 to biobased polymers. It has also been shown that biobased polymers can be used to design complex nanoparticle assembly structures including clusters,22, 23 networks,24-26 and films.27-29
The aforementioned structures can be formed in-situ (via sol-gel chemistry or hydrothermal/solvothermal methods)30-33 or by simply blending the nanoparticles and matrix.34-36 These nanostructures can afford new and unique properties to the biobased polymer matrix, together lessening environmental impact and fulfilling the necessary performance metrics.
In this report, we take advantage of the morphology of biobased polymers and design unique nanoassembly structures for producing high-performance biobased coatings. Two examples are demonstrated here: transparent UV-blocking coating and superhydrophobic coating. Starch and cellulose with hydroxyethyl modifications (HEC and HES) are used in the scope of this work. Though chemically identical, cellulose exhibits a rod-like morphology while starch is a coil. The conformation of the chain has monumental effects on the assembly of nanoparticles within the matrix, and the properties that result. The nanoassembly structures formed by silica (SiO2) and zinc oxide (ZnO) nanoparticles impart superhydrophobicity and UV-blocking properties (respectively) to the biobased polymer matrix. Therefore, polymer morphology can be used to develop highly performing green nanocomposites whose nanostructures grant them a competitive edge against traditional plastics.
Results
UV-Blocking Coating
The UV-blocking coating is composed of three elements: ZnO nanoparticle (30 nm, 0.8% wt.), biobased binder (HEC or HES) (4% wt.), and a chemical dispersant (Tween 20) (0.5% wt.). ZnO was chosen here for its unique band gap, which promotes UV-blocking in the UVA range.6
Using small nanoparticles helps produce a transparent UV-blocking film. It was found that the choice of polymeric binder drastically changes the UV-blocking capacity of the coating, with HEC largely outperforming HES (Figure 1) with impressive visible transparency.7
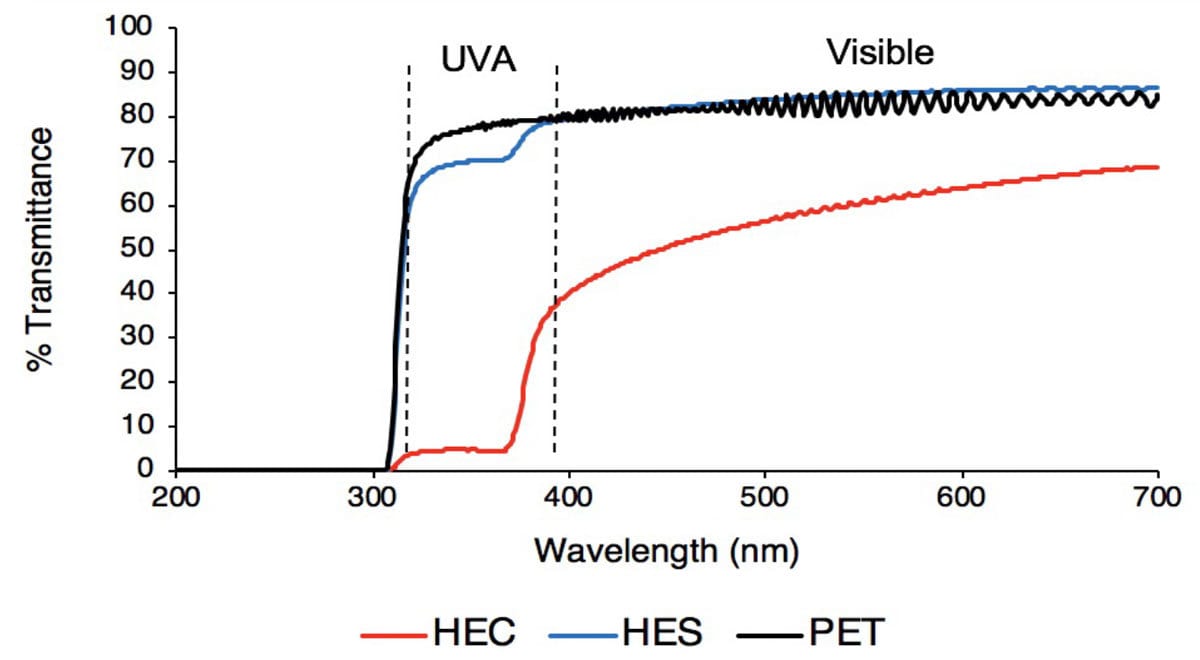
FIGURE 1 ǀ Comparison of UV Vis spectrum for the polyethylene terephthalate (PET) substrate alone, PET substrate coated with ZnO-HEC coating, and PET substrate coated with ZnO-HES coating.
SEM revealed the presence of loosely branched particle assemblies with HEC, and small, dense aggregates with HES (Figure 2), suggesting that the functionality of the coating is highly dependent on nanoparticle aggregation pattern. This result is surprising considering the similarities between polymer molecular structures. HEC and HES are chemically identical, only differing by their intermolecular bonds. HES takes on a coiled structure due to its cis glycosidic linkage. HEC, on the other hand, is rod-like as a result of its trans bonds (Figure 2). The variation in polymeric structure clearly impacts on the assembly of nanoparticles within the matrix.
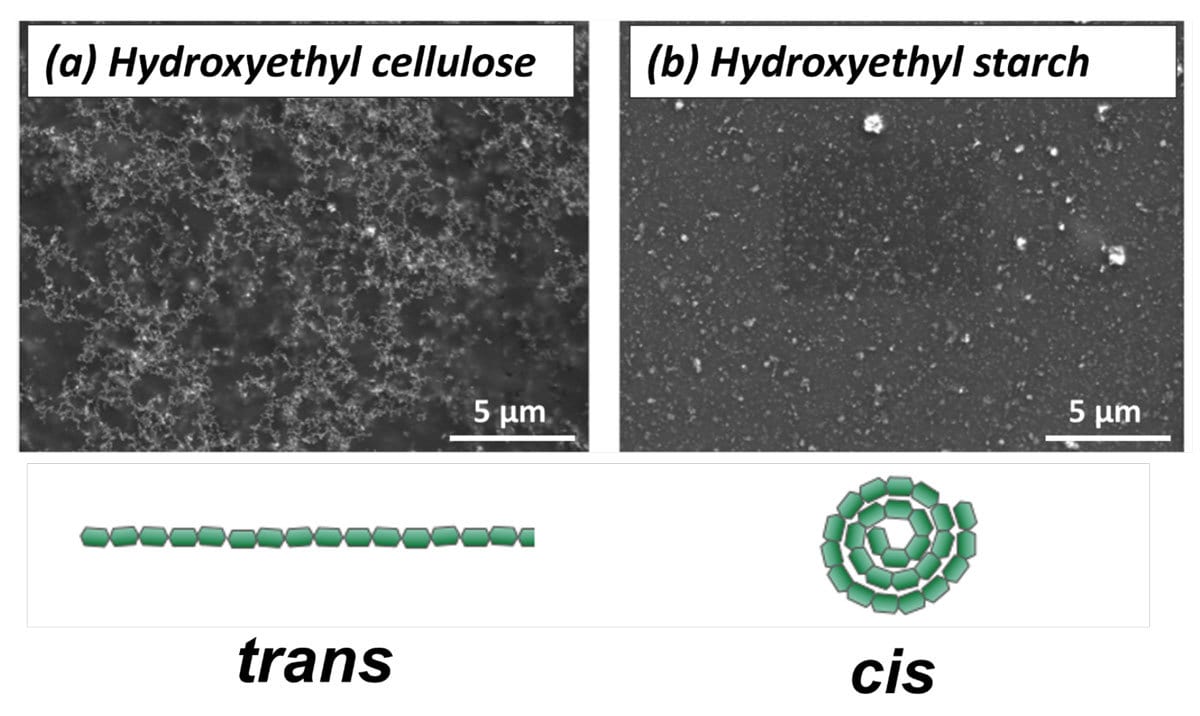
FIGURE 2 ǀ SEM micrographs of the aggregation patterns formed by the ZnO nanoparticles with binder polymers and their corresponding molecular structures: (a) HEC and (b) HES. Scale bar is 5 µm.
Superhydrophobic Coating
Silica dioxide nanoparticles form similar structures with HEC and HES at the same particle loading. However, when larger quantities of silica (3% wt.) are introduced in place of zinc oxide in the HEC and HES polymer systems, new capabilities were introduced to the coating system. Upon vaporized treatment of fluorinated silane on the dried coating surface, superhydrophobicity was achieved. Without nanoparticles, the silane treatment is shown to form small clusters on the polymer surface. These clusters appear larger in HEC than in HES. When nanoparticles are included in the treated coating, the cellulose polymer introduces multiscale roughness (with branched features), while the starch matrix only disperses the particles. The roughness post silane treatment with and without nanofiller is quantified via confocal and AFM techniques, respectively (Figure 3).
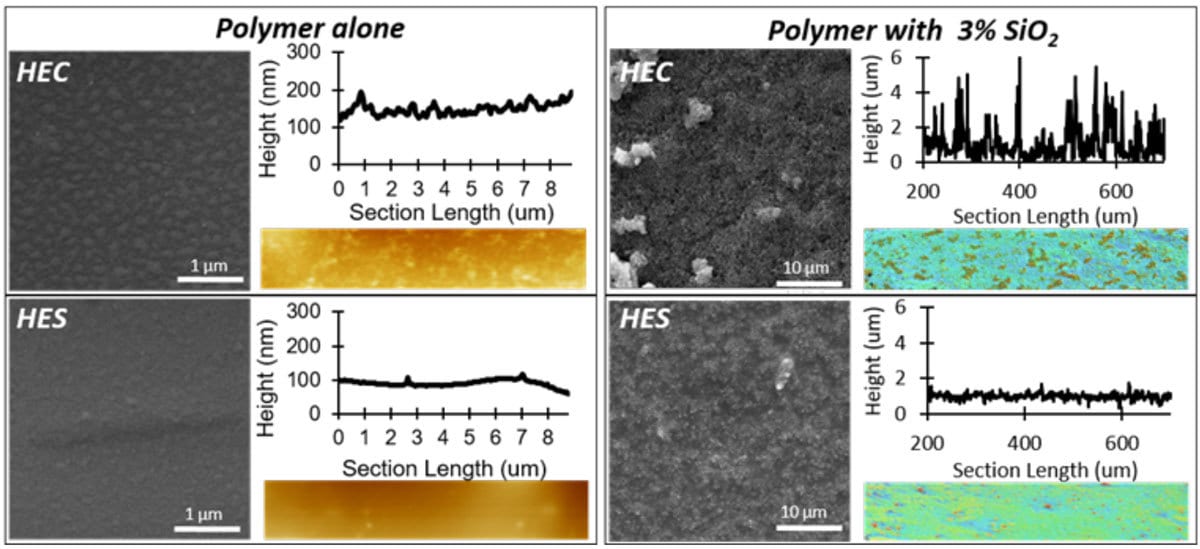
FIGURE 3 ǀ SEM micrographs of fluoro silane-treated composites of HEC and HES, with and without silica nanofiller, accompanied by the roughness profiles via AFM and confocal microscopy.
The roughness and assembly features largely alter the water repellency of the composite, with HEC achieving a 160° contact angle, outperforming HES by 20°. This is a significant improvement compared to the polymer without nanofiller (Figure 4). The structures formed by HEC are more robust than the HES derivative, sustaining the water repellency even after being immersed in water for 1 day.
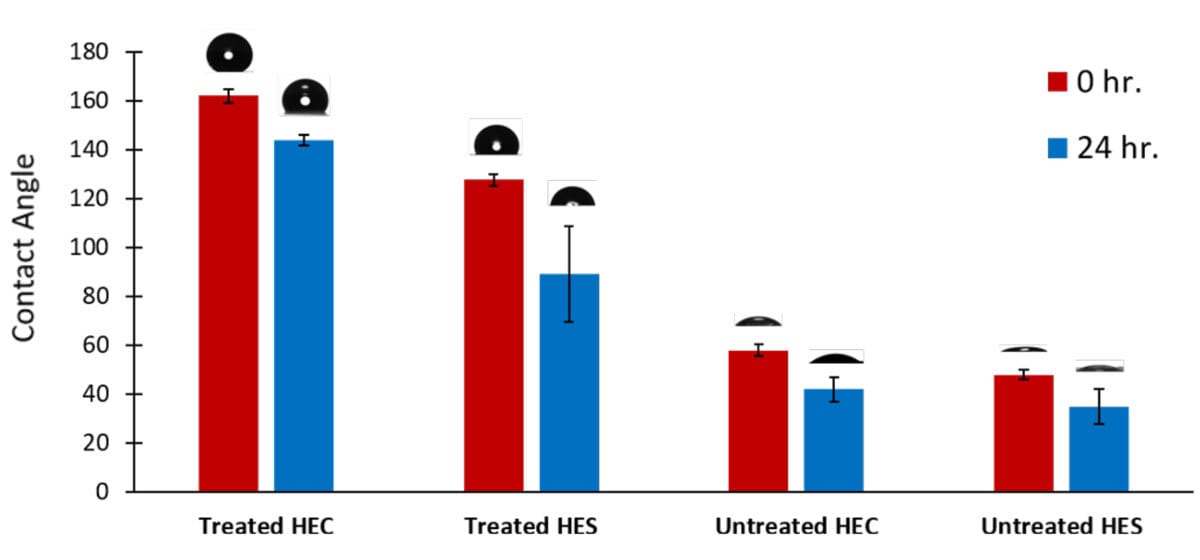
FIGURE 4 ǀ Contact angle measurement of HEC and HES films with and without silica nanoparticles, and the performance post immersion in water for 24 hrs.
The network structure formed by HEC has also been demonstrated to enhance the adhesion of the coating to the substrate. Even after immersion, the silane-treated HEC-silica coating is robust to adhesion testing via the crosshatch adhesion test (Figure 5). The untreated HEC shows adhesive strength prior to immersion, but after immersion little coating remains to be tested. HES, on the other hand, demonstrates poor adhesion, even after silane treatment (Figure 5).
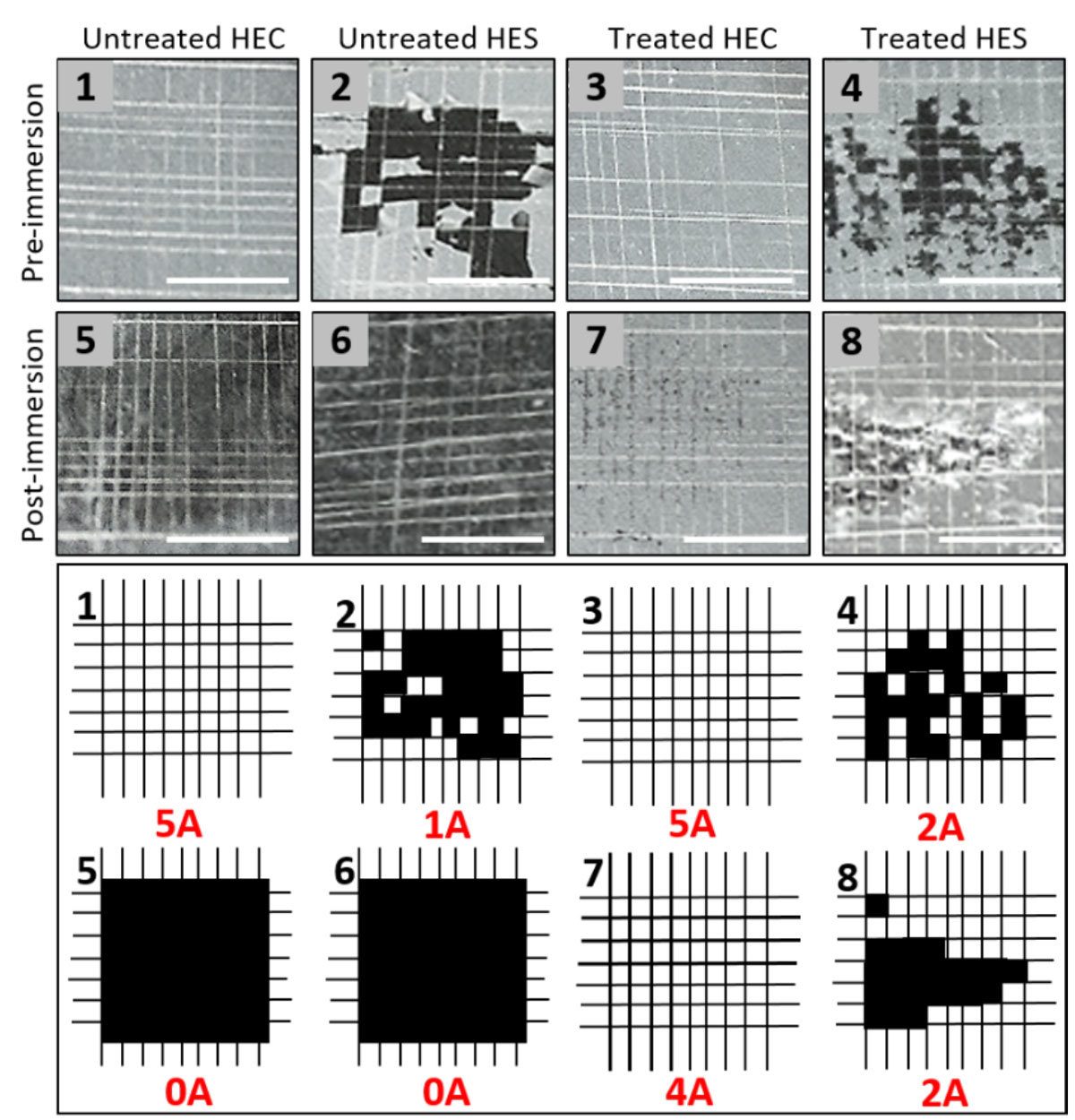
FIGURE 5 ǀ Crosshatch adhesion test of HEC and HES coatings with silica nanoparticles, and corresponding cartoons of the result. Coatings with and without silane treatment were tested, in addition to water immersion conditions.
Performance Highlights
Polymer chain conformation was used to mediate unique assembly structures of nanoparticles. The rod-like conformation of HEC formed a porous branched network, and the coil-like conformation of HES formed a dispersion of aggregates. The network assembly yielded a number of favorable properties based on nanoparticle selection.
• ZnO nanoparticles with HEC
- Highly efficient UV-blocking (95%) while maintaining 80% visible transparency
- Ultrathin thickness of 200 nm
• SiO2 nanoparticles with HEC
- Superhydrophobicity due to multiscale roughness from the silane treatment and nanoassembly pattern
- Maintained performance post immersion in water
- Strong adhesion post crosshatch test
Conclusion
Through this study, we demonstrate that biobased polymer morphology can be used to influence nanoparticle assembly structures. High-performing coatings can be fabricated with water-dispersible biobased polymers. The results bridge the assembly structures at the nanoscale, influenced by molecular conformation of biobased polymers, to the coating performance at the macroscopic level. Cellulose-derived biobased polymers HEC has created a unique network structure of nanoparticle assemblies, which has proven to be beneficial to enhance the functionality and performance of biobased coatings. Superhydrophobicity and adhesive strength are demonstrated with silica nanoparticles and UV blocking with zinc oxide nanoparticles. In addition, these structures are generalizable to different types of nanoparticles, which may inspire more innovative coating designs in future. Through this study we unveil new opportunities in economical and sustainable development of high-performance biobased materials. The potential applications may impact broad fields such as medical, civil, automotive and aerospace.
References
1 He, P.; Chen, L.; Shao, L.; Zhang, H.; Lu, F. Municipal Solid Waste (MSW) Landfill: A Source of Microplastics? -Evidence of Microplastics in Landfill Leachate. Water Res. 2019, 151, 38-45.
2 Sharuddin, S.; Abnisa, F.; Daud, W.W.; Arauda, M. K. A Review on Pyrolysis of Plastic Wastes. Energy Convers. 2016, 115, 308-326.
3 Eckhouse, S. U.S. Food Waste Challenge. USDA.gov (accessed May 15).
4 Silva, F.A.G.S.; Dourado, F.; Gama, M.; Poças, F. Nanocellulose Bio-Based Composites for Food Packaging. Nanomaterials 2020, 10, 2041.
5 Tian, M.; Zhen, X.; Wang, Z.; Zou, H.; Zhang, L.; Ning, N. Bioderived Rubber – Cellulose Nanocrystal Composites with Tunable Water-Responsive Adaptive Mechanical Behavior. ACS Appl. Mater. Inter. 2017, 9, 6482–6487.
6 Gidley, M.J.; Bociek, S.M. Carbon-13 CP/MAS NMR Studies of Amylose Inclusion Complexes, Cyclodextrins, and the Amorphous Phase of Starch Granules: Relationships Between Glycosidic Linkage Conformation and Solid-State Carbon-13 Chemical Shifts. J. Am. Chem. Soc. 1988, 110, 3820-3829.
7 Park, H.M.; Misra, M.; Drzal, L.T.; Mohanty, A.K. “Green” Nanocomposites from Cellulose Acetate Bioplastic and Clay: Effect of Eco-Friendly Triethyl Citrate Plasticizer. Biomacromolecules 2004, 5, 2281-2288.
8 Takeda, Y.; Hizukuri, S.; Takeda, C.; Suzuki, A. Structures of Branched Molecules of Amyloses of Various Origins and the Molar Fractions of Branched and Unbranched Molecules. Carbohydr. Res. 1987, 165, 139-145.
9 Hoover, R., Composition, Molecular Structure, and Physicochemical Properties of Tuber and Root Starches: a Review. Carbohydr. Polym. 2001, 45, 253-267.
10 Chung, H.J.; Liu, Q.; Donner, E.; Hoover, R. Composition, Molecular Structure, Properties, and In Vitro Digestibility of Starches from Newly Released Canadian Pulse Cultivars. Cereal Chem. 2008, 85, 471-479.
11 Hoover, R.; Ratnayake, W.S. Starch Characteristics of Black Bean, Chick Pea, Lentil, Navy Bean and Pinto Bean Cultrivars Grown in Canada. Food Chem. 2002, 78, 489-498.
12 Tankhiwale, R.; Bajpai, S.K. Silver-Nanoparticle-Loaded Chitosan Lactate Films with Fair Antibacterial Properties. J. Appl. Polym. 2009, 115, 1894-1900.
13 Jung, R.; Kim, Y.; Kim, H.S.; Jin, H.J. Antimicrobial Properties of Hydrated Cellulose Membranes with Silver Nanoparticles. J. Biomater. Sci. 2012, 20, 311-324.
14 Usman, A.; Hussain, Z.; Riz, A.; Khan, A.N. Enhanced Mechanical, Thermal and Antimicrobial Properties of Poly (Vinyl Alcohol)/Graphene Oxide/Starch/Silver Nanocomposites Films. Carbohydr. Polym. 2016, 153, 592-599.
15 Rahman, O.U.; Kashif, M.; Ahmad, S. Nanoferrite Dispersed Waterborne Epoxy-Acrylate: Anticorrosive Nanocomposite Coatings. Prog. Org. Coat. 2015, 80, 77-86.
16 Njoku, D.I.; Cui, M.; Xiao, H.; Shang, B.; Li, Y. Understanding the Anticorrosive Protective Mechanisms of Modified Epoxy Coatings with Improved Barrier, Active and Self-Healing Functionalities: EIS and Spectroscopic Techniques. Sci. Rep. 2017, 7, 15597-15609.
17 Khan, A.; Khan, R. A.; Salmieri, S.; Tien, C. L.; Riedl, B.; Bouchard, J.; Chauve, G.; Tan, V.; Kamal, M. R.; Lacroix, M. Mechanical and Barrier Properties of Nanocrystalline Cellulose Reinforced Chitosan Based Nanocomposite Films. Carbohydr. Polym. 2012, 90, 1601-1608.
18 Noorbakhsh-Soltani, S.M.; Zerafat, M.M.; Sabbaghi, S. A Comparative Study of Gelatin and Starch-Based Nano-Composite Films Modified by Nano-Cellulose and Chitosan for Food Packaging Applications. Carbohydr. Polym. 2018, 189, 48-55.
19 Ni, S.; Zhang, H.; Godwin, P.M.; Dai, H.; Xiao, H. ZnO Nanoparticles Enhanced Hydrophobicity for Starch Film and Paper. Mater. Lett. 2018, 230, 207-210.
20 Milionis, A.; Ruffilli, R.;Bayer, I.S. Superhydrophobic Nanocomposites From Biodegradable Thermoplastic Starch Composites (Mater-Bi®), Hydrophobic Nano-Silica and Lycopodium Spores RSC Adv. 2014, 4, 34395-34404.
21 Indumathi, M.P.; Sarojini, K.S.; Rajarajeswari, G.R., Antimicrobial and Biodegradable Chitosan/Cellulose Acetate Phthalate/ZnO Nano Composite Films with Optimal Oxygen Permeability and Hydrophobicity for Extending the Shelf Life of Black Grape Fruits. Int. J. Biol. Macromol. 2019, 132, 1112-1120.
22 Zhou, J.J.; Wang, S.Y.; Gunasekaran, S. Preparation and Characterization of Whey Protein Film Incorporated with TiO2 Nanoparticles. J. Food Sci. 2009, 74, N50-N56.
23 Hakim, R.H.; Cailloux, J.; Santana, O O.; Bou, J.; Sanchez-Soto, M.; Odent, J.; Raquez, J. M.; Dubois, P.; Carrasco, F.; Maspoch, M.L. PLA/SiO2 Composites: Influence of the filler modifications on the morphology, crystallization behavior, and mechanical properties J. Appl. Polym. 2017, 134, 45367.
24 Trinh, L.T.T.; Kjoniksen, A.L.; Zhu, K.; Knudsen, K.D.; Volden, S.; Glomm, W.R.; Nystom, B. Slow Salt-Induced Aggregation of Citrate-Covered Silver Particles in Aqueous Solutions of Cellulose Derivatives. Colloid Polym. Sci. 2009, 287, 1391.
25 Liu, L.; Wang, L.; Luo, S.; Qing, Y.; Yan, N.; Wu, Y. Chiral Nematic Assemblies of Silver Nanoparticles in Cellulose Nanocrystal Membrane with Tunable Optical Properties. J. Mater. Sci. 2019, 54, 6699-6708.
26 Feckl, J.M.; Haynes, A.; Bein, T.; Fattakhova-Rohlfing, D. Thick Titania Films with Hierarchical Porosity Assembled from Ultrasmall Titania Nanoparticles as Photoanodes for Dye Sensitized Solar Cells. New J. Food Chem. 2014, 38, 1996-2001.
27 Tang, B.; Wang, J.; Xu, S.; Afrin, T.; Tao, J.; Xu, W.; Sun, L.; Wang, X. Function Improvement of Wool Fabric Based on Surface Assembly of Silica and Silver Nanoparticles, Chem. Eng. J. 2012, 185, 366-373.
28 Liu, Z.; Li, M.; Turyanska, L.; Makarovsky, O.; Patane, A.; Wu, W.; Mann, S. Self-Assembly of Electrically Conducting Biopolymer Thin Films by Cellulose Regeneration in Gold Nanoparticle Aqueous Dispersions. Chem. Mater. 2010, 22, 2675-2680.
29 Jebel, F.S.; Almasi, H. Morphological, Physical, Antimicrobial and Release Properties of ZnO Nanoparticles-Loaded Bacterial Cellulose Films. Carbohydr. Polym. 2016, 149, 8-19.
30 Fu, F.; Li, L.; Liu, L.; Cai, J.; Zhang, Y.; Zhou, J.; Zhang, L. Construction of Cellulose Based ZnO Nanocomposite Films with Antibacterial Properties through One-Step Coagulation, ACS Appl. Mater. Inter. 2015, 7, 2597–2606.
31 Shankar, S.; Rhim, J.W.; Won, K. Preparation of Poly(lactid)/Lignin/Silver Nanoparticles Composite Films with UV Light Barrier and Antibacterial Properties. Int. J. Biol. Macromol. 2018, 107, 1724-1731.
32 Alonso, B.; Belamie, E. Chitin-Silica Nanocomposite by Self-Assembly. Angew. Chem. Int. Ed. 2010, 49, 8201-8204.
33 Yang, W.; Tian, H.; Liao, J.; Wang, Y.; Liu, L.; Zhang, L.; Lu, A. Flexible and Strong Fe3O4/Cellulose Composite Film as Magnetic and UV Sensor. Appl. Surf. Sci. 2020, 507, 145092.
34 Lee, T.W.; Lee, S.E.; Jeong, Y.G. Highly Effective Electromagnetic Interference Shielding Materials based on Silver Nanowire/Cellulose Papers. ACS Appl. Mater. Inter. 2016, 8, 13123-13132.
35 Cheng, Z.; Ma, Y.; Yang, L.; Cheng, F.; Huang, Z.; Natan, A.; Li, H.; Chen, Y.; Cao, D.; Huang, Z.; Wang, Y.H.; Liu, Y.; Yang, R.; Zhu, H. Plasmonic‐Enhanced Cholesteric Films: Coassembling Anisotropic Gold Nanorods with Cellulose Nanocrystals. Adv. Opt. Mater. 2019, 7, 1801816.
36 Saxena, N.; Naik, T.; Paria, S. Organization of SiO2 and TiO2 Nanoparticles into Fractal Patterns on Glass Surface for the Generation of Superhydrophilicity. J. Phys. Chem. C. 2017, 121, 2428-2436.